
Circadian Clocks
The circadian clock adapts organisms to the environmental day-night cycle both behaviorally and physiologically. In animals, not only are complex behaviors such as sleep and mood governed by this oscillator, but also different body functions such as digestion, circulation, and respiration. The basic mechanism of this clock is cell-autonomous in all studied species possessing a circadian clock i.e each cell contains a network of genes and proteins that can generate an oscillator with a period of approximately 24 hours. These oscillators can, in turn be entrained, to the relevant 24-hour environmental day-night cycle.In mammals, individual clocks in most cells are synchronized by a brain “master clock” in the suprachiasmatic nucleus of the hypothalamus. This orchestrates all rhythmic physiology and signal from this master clock entrain the clocks i peripheral cells. On a cellular level, circadian physiology extends even to processes such as proliferation, apoptosis, and DNA damage repair, which are thought to play important roles in cancer control. This global system is often referred to a the Circadian Timing System.
In plants there is no such "master clock" but the cellular circadian clock is entrained by the cycles of light and dark and temperature. The light input pathway to the clock is complex and only just becoming understood.
In individual cells, the circadian clock mechanism consists of oscillating feedback loops of transcription of “core” oscillator genes and posttranslational modifications of their protein products that regulate protein stability, activity, and/or localization.
Mammalian Clock
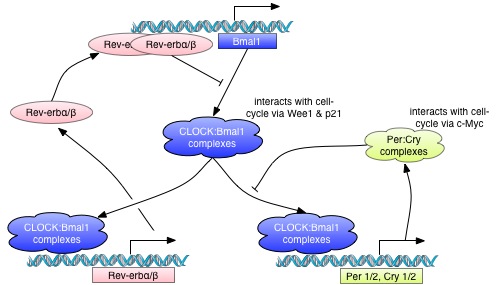
Recent models consider the clock a biochemical and cellular oscillator, and also a genetic network. A highly conserved negative feedback loop was discovered and elucidated at biochemical, cellular, and organismal levels in mammals and Drosophila; transcription factors play a prominent role in the clock mechanism. The bHLH-PAS transcriptional activators BMAL1 (official name: ARNTL, an ortholog of the Drosophila cyc gene) and its heterodimeric partner CLOCK (an ortholog of the Drosophila Clk gene) interact to bind to E-box cis elements present in the promoter regions of their target genes. These targets include two families of repressor proteins, the PERIODs (PER1, PER2, and PER3) and the CRYPTOCHROMEs (CRY1 and CRY2), which interact in a protein complex that translocates from the cyto- plasm to the nucleus. In the nucleus, this repressor complex physically associates with the BMAL1-CLOCK complex to inhibit E-box-mediated transcription. This process results in the cyclic transcription of these repressor genes, as well as thousands of transcriptional output genes elsewhere in the genome (Hughes et al., 2009; Panda et al., 2002a; Ueda et al., 2002).
Circadian Timing System
A comprehensive appraisal of the CTS for designing chronotherapy schedules
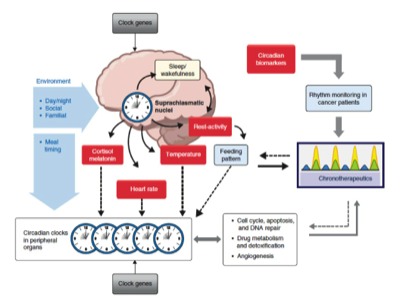
Plant Clock
For plants, which are sessile, the circadian clock is particularly important to synchronise internal metabolic and physiological processes with the external environmental conditions, and has been shown to increase fitness (Dodd et al 2005).
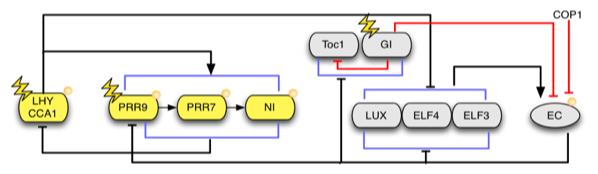
Figure. This shows the latest published network model (Pokhilko, 2012).
The clock network in Arabidopsis comprises a set of morning-specific genes, including the MYB transcription factors, LATE ELONGATED HYPOCOTYL (LHY) and CIRCADIAN CLOCK ASSOCIATED-1 (CCA1), and the pseudo-response regulators PRR5, 7 and 9. These act to regulate expression of a set of evening genes, encoding another pseudo response regulator (PRR1, also known as TOC1), GIGANTEA (GI), and three components of an Evening Complex, LUX ARRHYTHMO (LUX), and EARLY FLOWERING 3 AND 4 (ELF3 AND ELF4). CCA1[KD1] and LHY are coexpressed at dawn and are thought to repress expression of evening-specific components by binding to Evening Elements (EEs) in their promoters. Expression of LHY and CCA1 is then suppressed until the following morning through consecutive waves of expression of PRR9, 7, 5 and TOC1. Expression of LHY and CCA1 only resumes when this repression is lifted through downregulation of TOC1 and other PRRs by the evening complex. A mathematical model describing this process has been developed {polkhilko, 2012}.
ROBUST Project
Funding: BBSRC
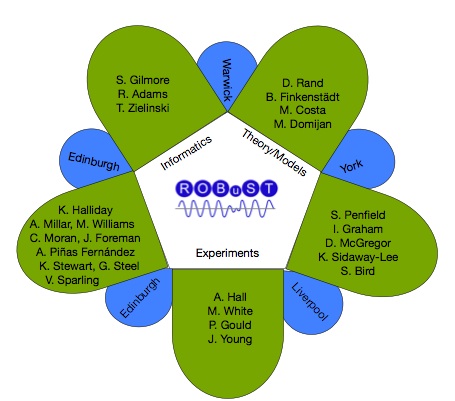
Robustness, sensitivity and plasticity
A major goal of systems biology is to understand the biological basis of network robustness, sensitivity and plasticity. Together, these features allow the network to accommodate, respond or adapt to environmental perturbation. In this project we take a systems approach to measure, model and analyse how temperature in the physiological range for Arabidopsis, from 4oC to 27oC, affects the molecular network comprising the phytochrome photoreceptor (phy), circadian clock and cold acclimation pathways. We aim to provide the first detailed understanding of temperature responses across any complex biological signalling network.In nature, environmental inputs from light and temperature are strongly correlated with each other and with daily timing, so it is no surprise that their signalling networks are also closely intertwined.
Temperature and the circadian clock
The circadian clock is temperature-compensated (buffered), some phytochrome responses are temperature-compensated whereas others are temperature-sensitive, and the cold acclimation pathway is highly sensitive to low temperatures. The network profoundly affects plant growth and development within the physiological temperature range: perturbations of the network can be lethal. As ambient temperatures are changing on a global scale, our findings will guide predictions of how the vegetation component of the biosphere, including crops, will respond to climate change.ROBuST aims
We will quantify temperature effects on the abundance, localisation, interaction and function of over 50 RNAs and proteins in our network.In this interdisciplinary venture we will develop new methods that advance network analysis. The programme has strong training and outreach components. We will run regular systems biology workshops and will extend Systems expertise beyond our two Systems Biology Centres, CSBE (now SynthSys) and WSB, to high calibre labs that are currently without this type of support. This project will generate a bank of resources in the form of biological materials (e.g. constructs and transgenic lines), mathematical tools and simulation software that we will make available to the wider research community. Finally we will forge strong connections with industrial partners who have expertise in crop improvement, metabolic functional genomics, and technology development. We intend that these links will lead to productive long-term collaborations.
Objectives relevant to this reporting period
2. Measure or statistically estimate parameter values for many biochemical processes
3. Develop new methods of network analysis, model reduction and parameter estimation
4. Test and predict the temperature-dependence of signalling function, locating key temperature sensors in the models
5. Mutate and mis-express the key components to test and refine models of their function across temperatures
6. Establish broadly-applicable principles that may underlie the temperature control of many gene networks
7. Quantify the network's role in productivity and survival traits of whole plants
8. Provide Systems Biology training to RA's and four PhD students, linked to external PhD cohorts
9. Link our Systems Centres (CSBE and WSB) to two institutions without formal Systems Biology support
10. Provision of resources, including experimental materials and software tools
Some results.
The current results include:- Among the outputs is a manuscript "Network balance via CRY signalling controls the Arabidopsis circadian clock over ambient temperatures" about the temperature regulation of the plant circadian clock and its interaction with the light input pathways. Circadian clocks exhibit “temperature compensation”, meaning that they show only small changes in period over a broad temperature range. Several clock genes have been implicated in the temperature-dependent control of period in Arabidopsis. We show that blue light is essential for this, suggesting that the effects of light and temperature interact or converge upon common targets in the circadian clock. Our data demonstrate that two cryptochrome photoreceptors differentially control circadian period and sustain rhythmicity across the physiological temperature range. In order to test the hypothesis that the targets of light regulation are sufficient to mediate temperature compensation, we constructed a temperature-compensated clock model by adding passive temperature effects into only the light-sensitive processes in the model. Remarkably, this model was not only capable of full temperature compensation and consistent with mRNA profiles across a temperature range, but also predicted the temperature-dependent change in the level of LATE ELONGATED HYPOCOTYL (LHY), a key clock protein. Our analysis provides a systems level understanding of period control in the plant circadian oscillator.
- Another manuscript is "Model optimisation and the value of constraints when fitting to data." (Authors: Mirela Domijan and David Rand) We consider how to analyse the fit of complex high-dimensional models to constraints determined by data. We discuss how to determine the value of constraints and how to optimise the choice of constraints to fit to. We illustrate these ideas by considering some models of the plant circadian clock and NF-kappaB signalling system which have very large numbers of parameters.
- "Physiological temperature compensation preserves temporal shape." Classically, temperature compensation is about how well the free-running clock's period is preserved when the temperature is changed. However, selection acts on organisms with an environmentally entrained clock, not one that is free-running. It is therefore interesting to connect selectable properties of the clock in a physiological situation that would lead to the much studied versions of temperature compensation in these non-physiological conditions. We consider here the hypothesis that in fact the property that selection has acted on is the preservation of the temporal shape of the core mRNAs and proteins of the clock. We present experimental evidence that, in the case of the plant circadian clock, this is the case for the transcription rate. We also analyse theoretically why it is feasible to evolve such a complex trait in a reasonable time and relate this physiological temperature regulation to classical temperature compensation. As part of our analysis we present a new tool for extracting the shape of the transcription rate profile from a Luciferase time series.
- New software tools: CoAST, wavelet-based software with a user-friendly GUI, ReTROS, that reconstructs dynamic transcription from time series p:LUC data, and CovETS, that enables comparative analysis of timeseries data. We have achieved near completion of the ROBuST p:LUC collection, which represents the most comprehensive assembly of p:LUC reporter lines in a single (Col) accession.
- Inference on periodicity of circadian time series, (Biostatistics, manuscript under revision). Costa M.J., Finkenstädt B.F., Gould P.D, Foreman J., Halliday K.J., Hall A., Roche V., Lévi F., Rand D. Costa et al. describes the development of robust period estimation methodology that was motivated by persistent variation in biological data.
- Song YH, Smith RW, To BJ, Millar AJ, Imaizumi T. (2012) FKF1 conveys crucial timing information for CONSTANS stabilization in photoperiodic flowering. Science 336: 1045-1049. Online 25 May 2012. doi: 10.1126/science.1219644.
- Pokhilko A, Fernández AP, Edwards KD, Southern MM, Halliday KJ, Millar AJ. (2012) The clock gene circuit in Arabidopsis includes a repressilator with additional feedback loops. Mol Syst Biol. 8: 574. doi: 10.1038/msb.2012.
- Guerriero ML, Pokhilko A, Fernández AP, Halliday KJ, Millar AJ, Hillston J. (2012) Stochastic properties of the plant circadian clock. J R Soc Interface 9: 744-56. Online 31 Aug 2011.
- Chew YH, Wilczek AM, Williams M, Welch SM, Schmitt J, Halliday KJ. (2012) An augmented Arabidopsis phenology model reveals seasonal temperature control of flowering time. New Phytol. May;194(3):654-65. doi: 10.1111/j.1469-8137.2012.04069.x. Epub 2012 Feb 21.
- Significant improvement and extension of the SASSy software suite.

LHY Project
PIs: Isabelle A. Carré, Peter Stockley, Bärbel Finkenstadt, Sascha Ott, David Rand. Postdocs: Siren R. Veflingstad, Sally Adams.
Funding: BBSRC
LHY transcription factor
The LHY transcription factor functions as part of a small gene network that constitutes the oscillator mechanism of the circadian clock in higher plants. In Arabidopsis, its function overlaps with that of a related protein named CCA1, and both genes are expressed in the early morning. During the day, both proteins bind to the promoter of a third gene named TOC1 to repress its transcription. Reduced levels of LHY and CCA1 proteins in the evening allow expression of TOC1 to resume. Accumulation of the TOC1 protein late at night promotes transcription of LHY and CCA1, thus initiating another cycle (Figure 1). The LUX, ELF4, GI, PRR7 and PRR9 proteins mediate additional feedback loops converging onto the promoters of LHY and CCA1. LHY also plays a role downstream of the clock to control the expression of rhythmic “output” genes. In addition to the clock-associated genes TOC1, GI, PRR9, LUX, ELF4 and ELF3, known targets include the morning-specific gene CAB2 and the evening-specific gene CCR2.How does the rhythmic accumulation of LHY result in diverse expression patterns?
The purpose of the proposed research is to develop a detailed understanding of how the rhythmic accumulation of the LHY transcription factor results in varied temporal patterns of expression from its downstream targets.The following hypotheses will be tested:
- Whether the binding affinity of the LHY transcription factor for individual targets influences the timing of transcriptional activation;
- Whether interactions with other transcription factors influences the timing of transcriptional activation;
- Whether the timing of transcriptional activation is determined by recruitment of the transcription factor to the promoter;
- Whether mRNA degradation contributes to the timing of mRNA accumulation
- The genome-wide identification of LHY binding sites.
- Determination of LHY affinity for different classes of binding sites.
- Identification of cofactors that may modulate the timing of expression of LHY target genes.
- The acquisition of time-course data for LHY protein levels, binding of LHY to selected genomic targets, transcriptional activation of these targets and accumulation patterns of the cognate mRNAs.
- Development of a set of mathematical tools to search for the most probable regulatory logic to account for the
6 data, and to predict the effects of any alterations in this logic. - In vivo test of predictions using promoter constructs fused to a luciferase reporter gene.
Some results.
ChIP-seq experiments have been competed and analysed. Follow-up experiments validating the ChIP-seq and evaluating the functionality of the binding sites found have been completed. A radical revision of the network structure is being proposed based on this. New methods have been developed for probing the detailed mechanics associated with the motif of found binding sites which correlate these with the timing of expression for the given gene.
Plant Clock
For plants, which are sessile, the circadian clock is particularly important to synchronise internal metabolic and physiological processes with the external environmental conditions, and has been shown to increase fitness (Dodd et al 2005).
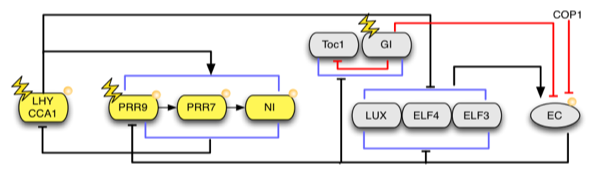
Figure. This shows the latest published network model (polkhilko, 2012).
The clock network in Arabidopsis comprises a set of morning-specific genes, including the MYB transcription factors, LATE ELONGATED HYPOCOTYL (LHY) and CIRCADIAN CLOCK ASSOCIATED-1 (CCA1), and the pseudo-response regulators PRR5, 7 and 9. These act to regulate expression of a set of evening genes, encoding another pseudo response regulator (PRR1, also known as TOC1), GIGANTEA (GI), and three components of an Evening Complex, LUX ARRHYTHMO (LUX), and EARLY FLOWERING 3 AND 4 (ELF3 AND ELF4). CCA1[KD1] and LHY are coexpressed at dawn and are thought to repress expression of evening-specific components by binding to Evening Elements (EEs) in their promoters. Expression of LHY and CCA1 is then suppressed until the following morning through consecutive waves of expression of PRR9, 7, 5 and TOC1. Expression of LHY and CCA1 only resumes when this repression is lifted through downregulation of TOC1 and other PRRs by the evening complex. A mathematical model describing this process has been developed {polkhilko, 2012}.

C5Sys Project
Funding: ERSSysBio+ & BBSRC
This project is particularly timely. On the one hand, remarkably little is known, to date about how these two crucial oscillators interact, how these interactions affect cellular proliferation in cancer, and how they could be best exploited for prevention and therapeutic purposes. On the other hand, the understanding of the component systems and the available biological, theoretical and analytical tools and techniques are at a level where a major breakthrough can be expected.
Circadian rhythms are generated by self-sustained intracellular molecular clocks, for which many of the key genes and transcriptional and posttranslational feedback loops have been identified. Modern genomics research has revealed that the molecular clock rhythmically controls the transcriptional activity of more than 10% of the genome, and drives circadian oscillations at the molecular, cellular and organism levels. Examples of clock-controlled biological processes include, the sleep-wake cycle, body temperature, hormone secretion, cell proliferation, metabolism and drug detoxification (1, 2, 24, 25). Mammals contain a master circadian clock, located in the suprachiasmatic nuclei (SCN) of the hypothalamus. The SCN clocks are reset daily by the environmental 24-h light-dark cycle, which, in turn synchronize clocks in peripheral organs to ensure coordination and optimal timing of key physiological processes (1, 2, 24, 25). The Circadian Timing System has therefore to be viewed as a hierarchical, mutiscale and fully integrated system.
Circadian Timing System
A comprehensive appraisal of the CTS for designing chronotherapy schedules
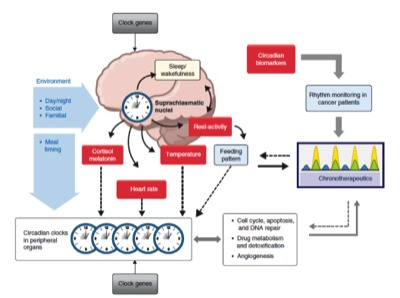
Mammalian Clock
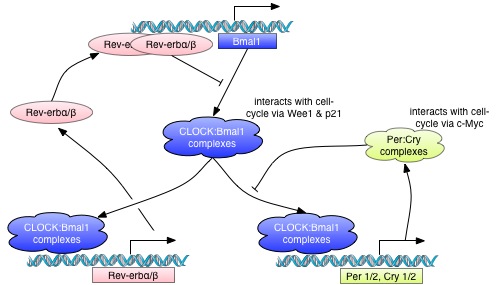
Recent models consider the clock a biochemical and cellular oscillator, and also a genetic network. A highly conserved negative feedback loop was discovered and elucidated at biochemical, cellular, and organismal levels in mammals and Drosophila; transcription factors play a prominent role in the clock mechanism. The bHLH-PAS transcriptional activators BMAL1 (official name: ARNTL, an ortholog of the Drosophila cyc gene) and its heterodimeric partner CLOCK (an ortholog of the Drosophila Clk gene) interact to bind to E-box cis elements present in the promoter regions of their target genes. These targets include two families of repressor proteins, the PERIODs (PER1, PER2, and PER3) and the CRYPTOCHROMEs (CRY1 and CRY2), which interact in a protein complex that translocates from the cyto- plasm to the nucleus. In the nucleus, this repressor complex physically associates with the BMAL1-CLOCK complex to inhibit E-box-mediated transcription. This process results in the cyclic transcription of these repressor genes, as well as thousands of transcriptional output genes elsewhere in the genome (Hughes et al., 2009; Panda et al., 2002a; Ueda et al., 2002).